Typically, if soil gets much attention from the general public, it is simply for how well (or poorly) plants grow in it. Soil science—an incredibly rich, complex and multifaceted academic discipline—has long recognized that Earth’s soils are a dynamic interaction of physical, chemical and biological properties. Yet most of us rarely give any thought to the fact that the ground beneath our feet is a complicated, ever-moving tangle of rocks and animals and plants and water and chemical compounds that rivals the ocean as a wild, dark, mysterious and inscrutable realm.
When we have focused on soil, it has often been to solve practical questions that arise: How do I keep this stuff from washing away? What’s the best way to till this dirt? What chemicals can I add so the tomatoes stop getting blossom-end rot?
Our understanding and thinking continues to change. Farmers, conservationists, scientists and others fascinated by soils have started pushing us all to ask questions about what lives in the soil. For the first time, there is a nationwide conversation about the paramount importance of soil biology.
It turns out this biology question is key to many environmental and economic questions of our time. Increasingly, we understand that healthy soils are productive and resilient, ultimately sustaining abundant crops with fewer costly inputs down the road. For reasons that we are just beginning to understand, the biology of certain soils can also suppress plant diseases, much in the same way a healthy gut biome in people might help prevent human diseases. There’s also mounting evidence that we can harness the incredible root systems of plants and their microbial allies to store vast quantities of atmospheric carbon dioxide in Earth’s soils at rates that could help offset human-generated greenhouse gas. And, as we continue to face a striking global loss of wild plants and animals, we are becoming more aware that soil is part of the fundamental ecology of all species—it provides a living platform for tigers and crickets, bacteria and bees, oaks and wildflowers, as well as the minerals that build not only the cells of those species but also our own.
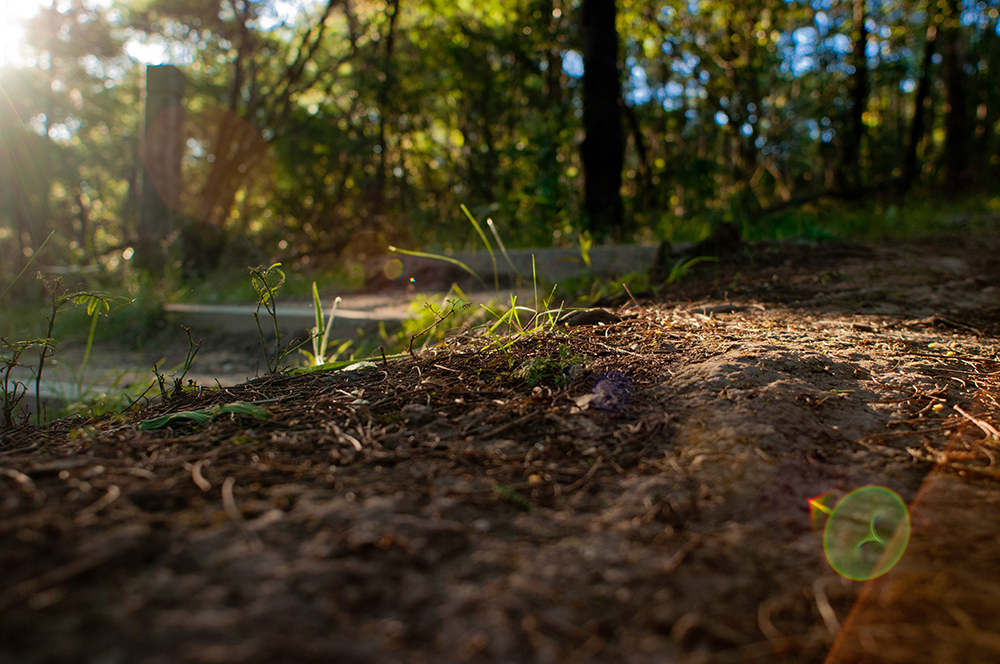
This publication is our addition to the discussion on soil biology. It is impossible to tell the story of every living species connected to the ground beneath us. With that in mind, we have focused this guide on the diverse, often overlooked, and essential living species that we know best: the major invertebrates (macrofauna and mesofauna) found in temperate agricultural soils. There is a focus on North America in the groups of organisms and the soil health practices that are covered, but many groups are present in these soil types around the world, and the same management principles apply. Larger soil animals, such as ground beetles, woodlice, and springtails, and their many companions, have received less attention than soil microbes in recent years. We hope this publication helps fill that gap.
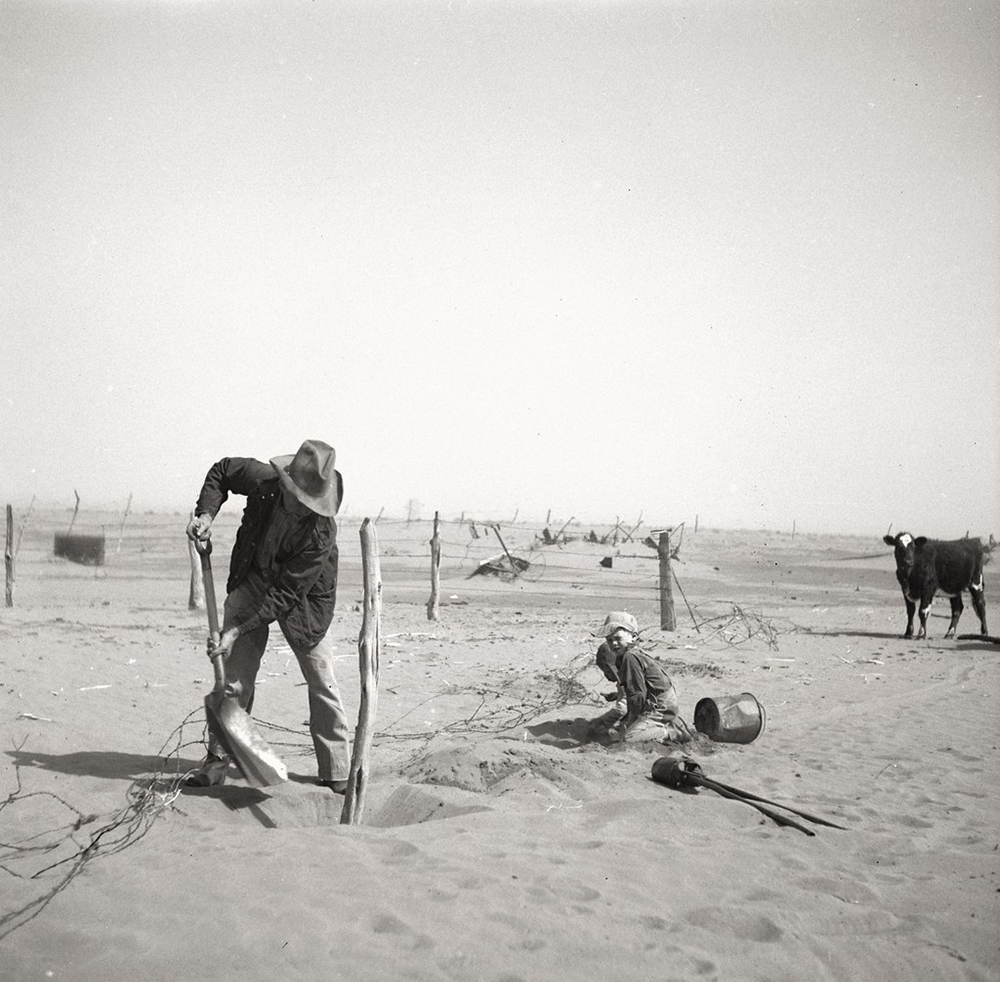
Finally, we acknowledge the many thoughtful soil conservation pioneers who laid the groundwork for this effort. Most notably, faced with the great Dust Bowl of the 1930s that threatened the entire economic and environmental foundation of the United States, we survived thanks to the innovative work of the U.S. Department of Agriculture (USDA) Soil Conservation Service (now the Natural Resources Conservation Service), as well as local conservation districts and individual farmers. We all owe that earlier generation of soil conservationists a debt of gratitude, just as we all owe you, the reader, our gratitude for taking up this same cause.
A Short History of Soil
Soil is being made continuously all around us through the slow decomposition and disintegration of larger mineral and carbon components. The most abundant of these objects, on a planetary scale, is the bedrock of Earth itself.
Bedrock is created across a timeframe as old as Earth’s. For example, the accumulated skeletons of ancient animals may become compressed in marine sediments to create limestone. The rise and fall of seas, movement of tectonic plates, eruption of volcanoes and other factors recycle and rearrange Earth’s geology into mountain ranges or eroded canyons. Flowing, freezing and thawing water; glacial movement; the chemistry of our atmosphere; the cycling of nutrients and energy through organisms in the soil; and other forces work to constantly disintegrate the upper layers of rock across the planet. In soil science, these forces are collectively referred to as weathering and are broadly divided into physical weathering (such as abrasion) and chemical weathering (such as oxidation). The weathered particles of all this rock (referred to as parent material) may remain as semi-stable fragments for long periods, or they may dissolve as minerals into water. Weathering creates smaller particles from the parent material. These particles are transported and deposited by gravity, water, wind and glaciers.
Of course, soil has organic origins along with mineral sources. Once rock is weathered into smaller particles, plant roots can grow within it, and this begins the transformation of mineral pieces into soil. This mixing, ongoing disintegration and recombination of organic and inorganic parent materials creates our soils. Most of those actions are dependent on (or at least are carried out most efficiently by) the life in our soils, especially fungi, plant roots, and—most dynamically—animals. Roots create an environment for microorganisms and mesofauna and, as organic matter builds up, for larger animals and plants. Some soil organic matter, such as peat, can be ancient, forming as dead vegetation that accumulates in wetlands, eventually filling up wetlands with thick, relatively stable organic residue that can persist for centuries. On upland locations, soil is constantly accumulating through the deposition of dead vegetation, dead animals and dead plant roots. Soils tell the stories of a landscape’s history.
The Functions of Soil
Soils provide many practical services that combine to make the world a livable place. Some of these services are so obvious that we tend to take them for granted, while others are mostly invisible and so large that they can be challenging to measure or document. These are among the most valuable things that soils provide us:
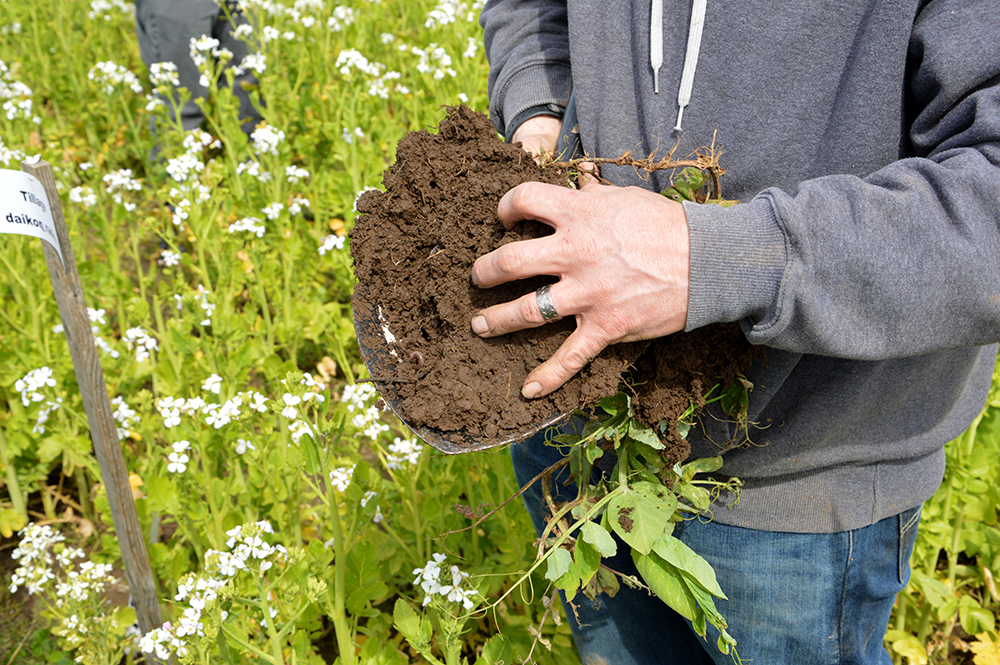
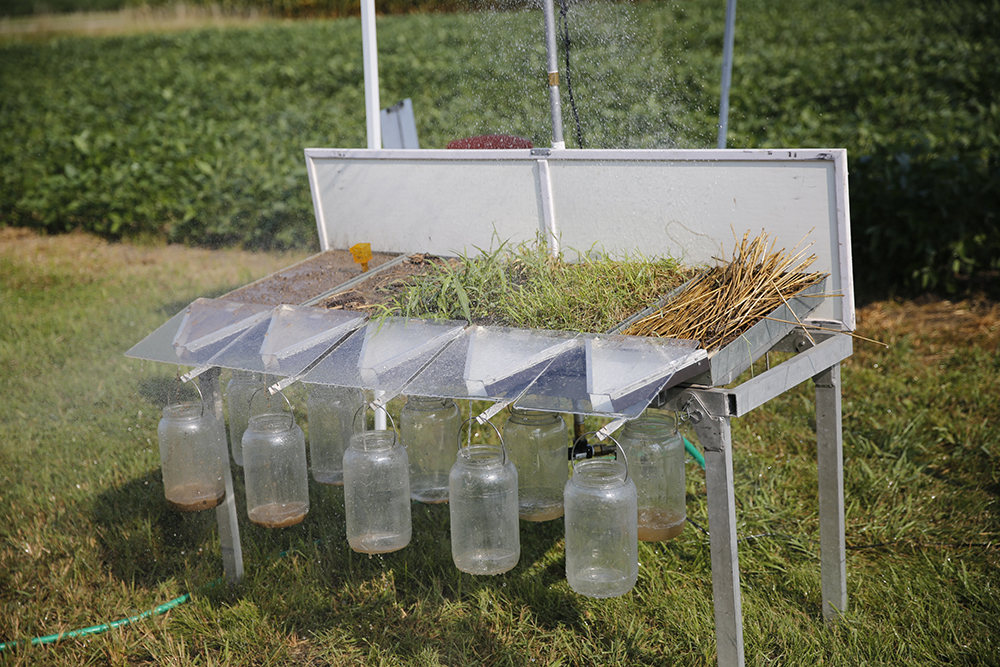
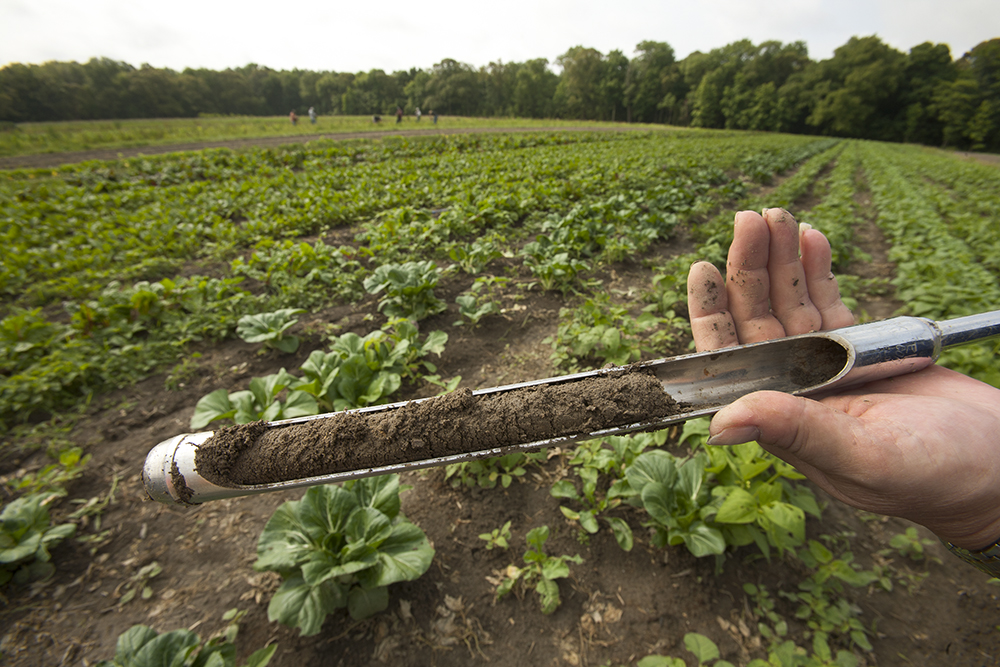
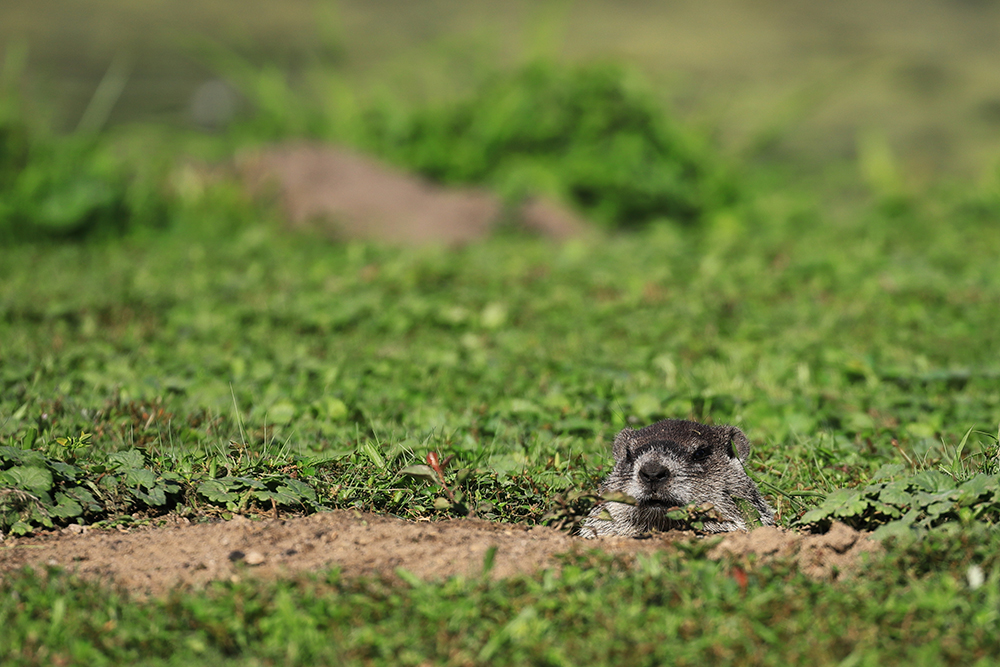
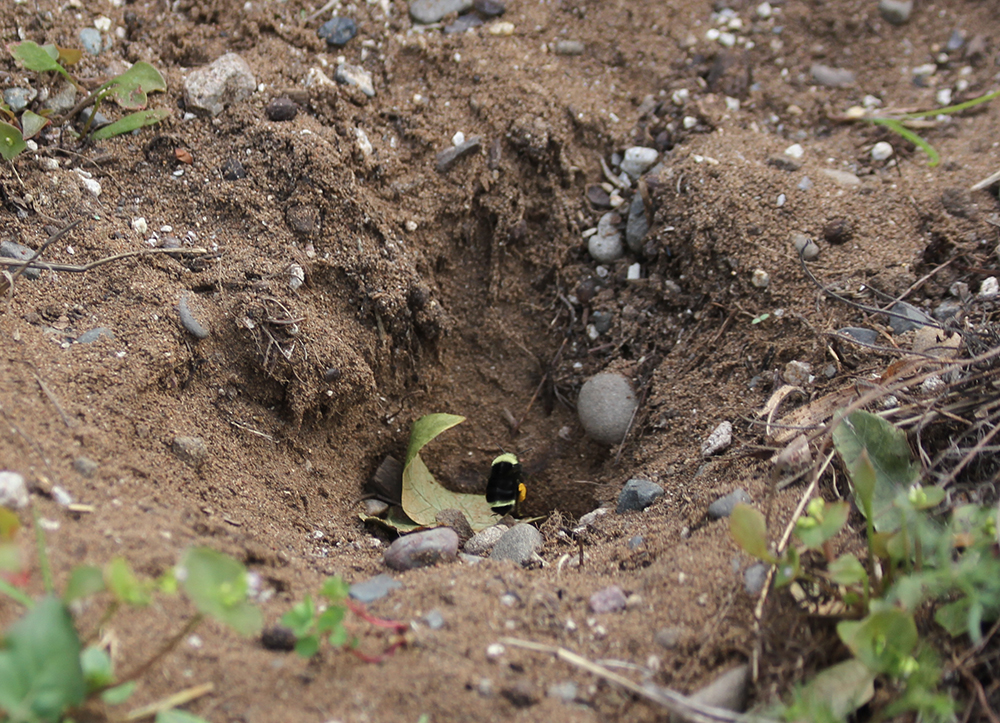
Figure 3—Soil provides many essential functions, including (clockwise from left): a medium in which plant life can grow (cover crop workshop), water supply and filtration (seen during this infiltration demonstration), recycling and storing organic matter (here a farmer uses a soil probe to check organic matter depth), and shelter for a variety of animals (like groundhogs and bumble bee colonies).
- A medium for plant growth: Soils not only provide a physical anchor for plant roots but also function as a sponge that absorbs water, air, and nutrients, slowly releasing those materials to sustain plant growth. Soil also protects plants from disease, pests and stress.
- Water-supply regulation and filtration: The infiltration of rainwater and snowmelt into soil is made possible by pores between soil particles and by openings created by plant roots and animal tunnels. Some of this water is retained in soil pores and even pulled upward against the force of gravity through capillary action. This spongelike property of soils, known as water-holding capacity, is variable depending on the texture of soils. By holding onto water, soils slow the water’s dispersal rate and reduce the potential for flooding. Soil is also the Earth’s largest natural water filter; as water passes through soil into groundwater reserves, various physical, chemical and biological processes filter contaminants—binding to, degrading or otherwise removing contaminants from groundwater used for drinking or irrigation.
- Recycling and storage of organic matter: Most terrestrial plants and animals ultimately become soil when they die. Decomposition by fungi, bacteria, insects and other soil organisms transforms once-living plants and animals into forms that can be used by other living things as energy sources for growth and maintenance. Some organic matter is also locked away into semi-stable forms of carbon that can remain in soil for centuries. This ability to sequester carbon remains one of the most attention-grabbing properties of soils and has the potential to play a major role in mitigating climate change.
- Habitat for wildlife: This guide is mostly about the insects and other invertebrate animals that live in soil; however, many animals—ranging from burrowing owls to tortoises to foxes—also nest, burrow or hibernate in soil. Even some very large animals, including bears and alligators, excavate underground dens. In many cases, secondary inhabitants, including creatures such as snakes or bumble bees (which might occupy tunnel systems excavated by rodents), move into burrows created by other animals. The impact that some of these animals have on soils is not often considered, yet the churning, mixing and rearranging of soils performed by animals can be significant. It’s estimated that in some areas, ants move more than a ton of soil per acre, per year. This tunneling activity moves organic matter on the soil surface below ground and brings subsoil to the surface, creating a mixed substrate and adding macropores that allow air and water movement within soil.
- An engineering medium: Soil is the foundation (or construction material) for roads as well as homes, bridges, buildings and countless other structures. Different types of soil have variable bearing strength, compressibility, seismic stability and potential to swell or shrink. These and other engineering considerations are often influenced by fundamental soil properties such as particle size, mineral types, bulk density and more.
How Soils Are Classified
Soil is the dynamic natural body of mineral and organic matter that covers terrestrial Earth. This body changes over time as climate and living organisms act upon the rocks and organic matter from which soil is created, called parental material.
Because climate and bedrock vary significantly across the globe, the resulting types of soil found on Earth also differ greatly from location to location. To help us understand and compare soil types, various systems of soil classification have been developed. These classification systems use a taxonomic hierarchy to categorize soils in specific locations, similar to the taxonomic hierarchies used to classify plants and animals.
In the case of soils, the classification hierarchy consists of distinct orders, suborders, great groups, subgroups, families, series and phases. Soil orders are the classification level for applied use. Orders can be based on age or weathering status (inceptisol, entisol, oxisol), reflect what grew there (mollisol, spodosol), or have been shaped by hydrological influences (aridisol, histosol). Farming occurs on all soil orders, but certain orders are better for production than others. Along with this classification system, soil scientists have created specialized terminology for describing soil architecture.
Setting aside formal soil classification, at a general level—such as when comparing different types of farm soils— we typically group soils according to texture based on the relative percentage of sand, clay and silt in any given soil sample. Each of these mineral particles is formally defined by a size range, and their specific combination in any given soil sample influences physical and chemical properties, such as bulk density, water-holding capacity, hydraulic conductivity, porosity, the exchange and absorption of ions, and more.
Along with soil texture, soil organic matter (SOM) influences soil properties and the way we categorize different soils. SOM is the fraction of the soil consisting of various plant and animal residues. There are several categories of SOM that differ in chemical composition and stage of decomposition, and the majority of SOM was originally plant residues. Some types of SOM are forms that are readily available food sources for soil microorganisms. Other types of SOM are long-lived and resistant to further breakdown. These store and release carbon over months and years. Management practices alter total organic carbon and the proportion of carbon present in each category. One aspect of farming for soil function and health is to understand how management practices affect SOM.
Finally, we often group soils by moisture categories. Very dry, well-drained soils may be described as xeric, while soils that are saturated with water for extended periods are known as hydric. Soils with a relatively even balance of moisture are called mesic and represent the primary soils used for agricultural production.
Soil Properties
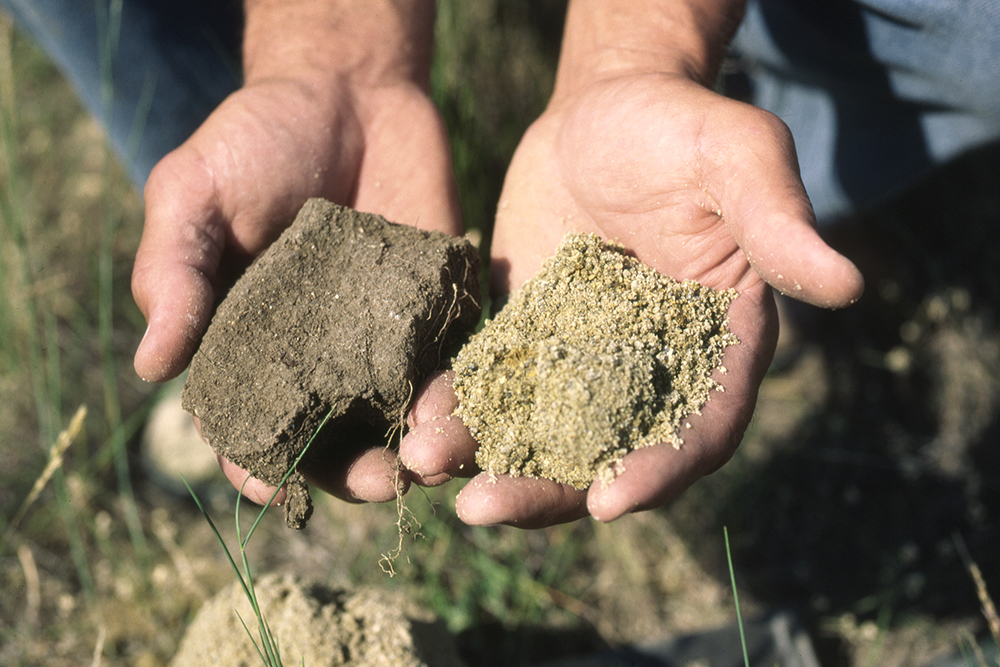
Soils are a fascinating mix of living and nonliving things. On some levels, they function as a superorganism—an enormous singular living thing made up of many smaller living things. In other ways, soils function as an amalgamation of rock and mineral particles. Soils are both alive and not alive at the same time. This condition provides us with an interesting mix of physical, chemical and biological properties that can be readily observed. This section provides a basic catalog of some of the most fundamental properties in each of those categories.
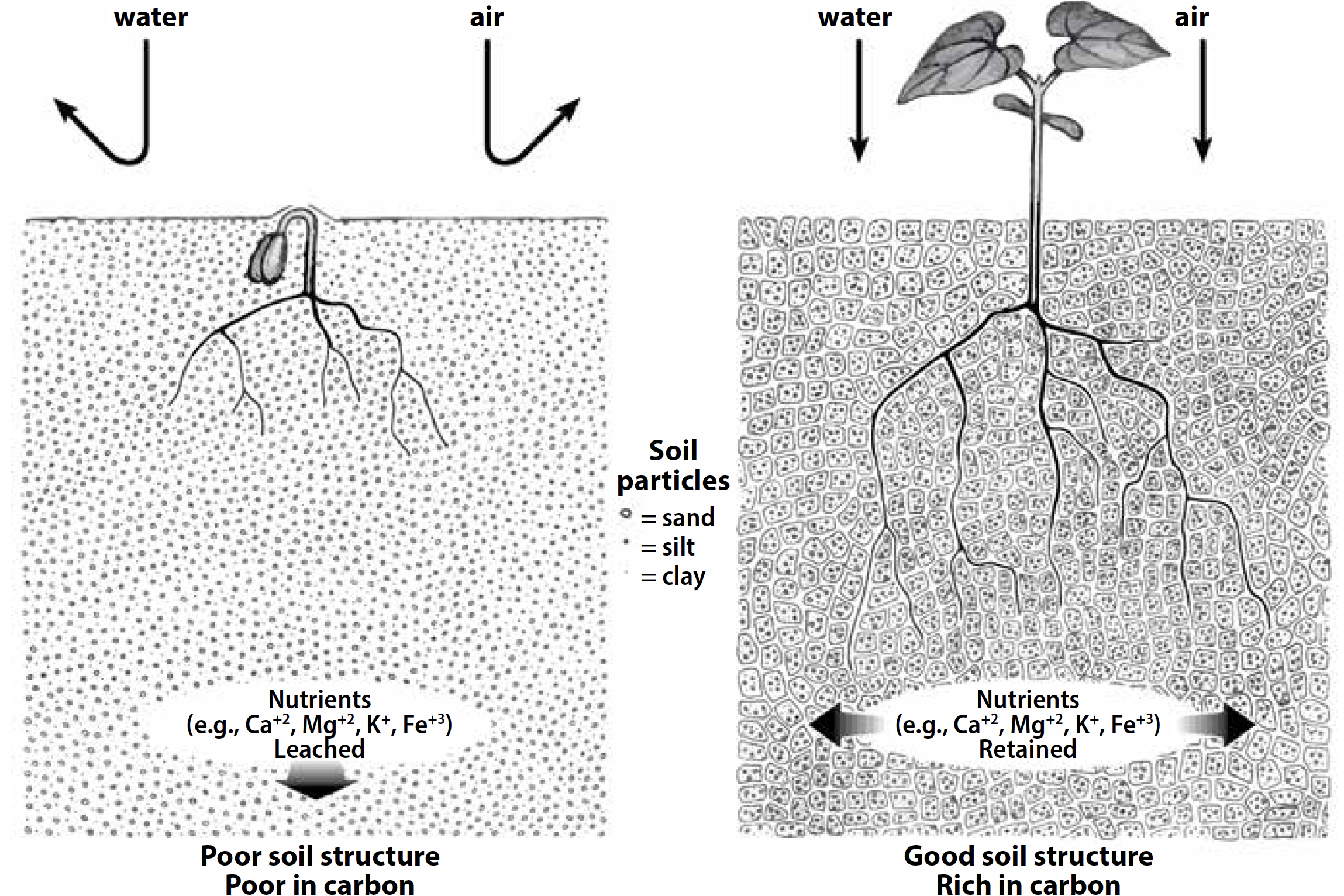
Physical Properties of Soil
- Texture: Nonorganic soil particles are classified by size into clay (small), silt (medium) and sand (large). Of these, clay has uniquely small pores and electric charges around its particles, which retain water and bind with nutrients. The ratio of these three particles relative to each other is a basic way of defining a soil’s type, such as a silt loam or a sandy loam.
- Structure and aggregates: Soil particles form aggregates, which are particles bound by organic or mineral compounds. Soils can be evaluated by how those aggregates hold together or resist deformation when crushed. Healthy soils have good aggregate stability—the soil resists compaction and the pore spaces that are important for root growth, animal movement, water infiltration and gas exchange remain intact. Powdery soil or soil with large, hard clods are examples of poor soil structure.
- Bulk density: The weight of soil relative to its volume is an indicator of how much pore space exists between the particles. This property is commonly measured in grams per cubic centimeter, with higher numbers reflecting less pore space. Because organic matter provides an abundance of pore spaces, even the addition of a small amount of organic matter can reduce the bulk density of a soil.
Biological Properties of Soil
- Nutrient cycling: One of the most important services that soil organisms perform is the decomposition of organic matter (such as dead plants and animals) into smaller pieces, which then have greater proportions of surface area. Mineralization is the further decomposition and transformation of organic matter into nutrients available for plants. This combined process, called nutrient cycling, especially the cycling of nitrogen and carbon, is a critical factor in soil fertility. Soil microbes have historically received most of the attention for nutrient cycling, yet larger organisms also play key roles. The roles of soil invertebrates can be categorized as: ecosystem engineers that create and maintain habitat; herbivores and predators that regulate the populations of other organisms; and primary decomposers that accelerate the decomposition process by breaking down plant or animal material into pieces more accessible to microorganisms.
- Microbial diversity and abundance: Each combination of soil characteristics, time, and conditions shape microbial communities. The composition of species that are present (diversity) and quantity of each (abundance) will drive different ecosystem services. For example, arbuscular mycorrhizae (types of fungi) and rhizobia (types of bacteria) make phosphorus and nitrogen more available to plant roots for uptake. Saprotrophic fungi cycle nutrients by breaking down leaf litter near the soil surface and mineralizing carbon.
The Nitrogen Cycle
Nitrogen is an essential chemical element for life on Earth and is present in many different compounds. The transformation and reuse of nitrogen in biological and geochemical processes is described in the nitrogen cycle.
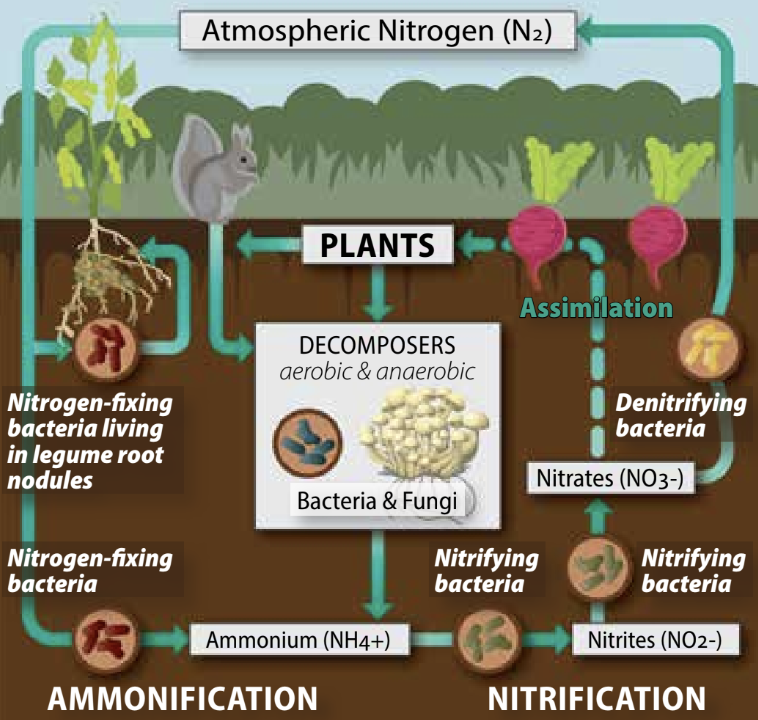
Bacteria and other microbes are the natural key in the terrestrial nitrogen cycle. Soil is the interface where nitrogen fixation occurs and where reactive forms of nitrogen are held and taken up by plants. Nearly 70% of the atmosphere is nitrogen gas (N2), but this form of nitrogen is nonreactive and is not biologically available to most life forms. For example, we humans take in nitrogen with each breath, but we exhale it. Our intake of usable nitrogen comes via the plant and animal ingredients in our food.
But how did it get from the atmosphere into plants and animals? We have bacteria to thank. Nitrogen-fixing bacteria grow in close symbiosis within specialized structures, called nodules, in the roots of certain plant species. The majority of plants in the bean, or legume, family (Fabaceae) share symbiotic relationships with one or more nitrogen-fixing bacteria.
Symbiotic nitrogen fixation also occurs in more than 30 non-legume plant genera. There are other groups of bacteria that live freely in the soil and also fix nitrogen. Nitrogen-fixing bacteria take in atmospheric nitrogen from soil pore spaces and convert it to organic nitrogen. Bacteria and fungi decompose dead plants or animals and also convert the nitrogen in those tissues to ammonia. Groups of nitrifying bacteria convert ammonia to ammonium, ammonium to nitrite (NO2-) and then nitrite to nitrate (NO3-), which is the form of nitrogen available for plants to take up. Denitrifying bacteria convert nitrate back to atmospheric nitrogen.
Apart from biological nitrogen fixation, additional energy can convert atmospheric nitrogen to biologically usable forms. High enough levels of energy to fix nitrogen occur naturally via lightning and through the manmade Haber-Bosch process, which uses high pressure, high temperatures, and natural gas.
Chemical Properties of Soil
- Cation-exchange capacity: Elements that occur in soil sometimes exist as positively charged ions, called cations. Aluminum, magnesium, potassium, calcium and hydrogen are some of the common cations found in soils. In contrast, small soil particles such as clay and organic matter have negatively charged surfaces that attract these cations, slowing the rate at which they are leached out of the soil by water. Despite this, some cations are inevitably lost or taken up by plants. When this happens, those lost cations may be replaced by others in a process called cation exchange. The measure of negative charges in a unit of soil that can rapidly exchange cations is called the cation-exchange capacity. (To put it another way, it is the measure of how many cations a soil sample can hold in an exchangeable form.) Typically, the greater the proportion of clay and organic matter in a soil, the greater the cation-exchange capacity will be, and the greater the potential will be for the soil to retain most plant nutrients.
- Soil pH: The acidity or alkalinity of a soil is known as the soil pH. This property is influenced by the parent material (or bedrock) from which the soil is created, as well as climate, vegetation and other factors such as fertility management and irrigation. For example, in the northeastern United States, leaching of basic cations is one of the primary causes of soil acidification. Various plant nutrients are soluble at different ranges of pH. For many agricultural plants, a slightly acidic soil in the pH range of 6.0 to 6.8 is often preferred. Inputs used to alter the pH of a soil, such as lime or sulfur, may influence the types and abundance of soil organisms.
Other Characteristics
The following characteristics are shaped by a combination of physical, chemical, biological, and temporal influences:
- Color: The parent material and iron, organic matter and other soil components create distinct soil colors that vary between locations and depths. Moreover, oxidation or other reactions can cause variations in soil color. In many cases, colors can provide clues to what materials are present in a specific soil sample, as well as whether a soil is well aerated, poorly drained or highly weathered.
- Horizonation: Soils form distinct layers, called horizons, that make up a deep cross section of soil, called a soil profile. Horizons usually run parallel to the ground surface. Soil horizons are often labeled with letters of the alphabet. The top horizons (O and A) consist of the organic material and small particles typically called topsoil. Deeper horizons (such as B, C and E) are typically made of minerals with little organic matter. Each horizon will typically have a distinct color and texture that sets it apart from the other horizons around it in a profile. (The horizon labeled O is typically found in forest soils and represents organic matter, but the other letters assigned to horizons are arbitrarily alphabetical.)
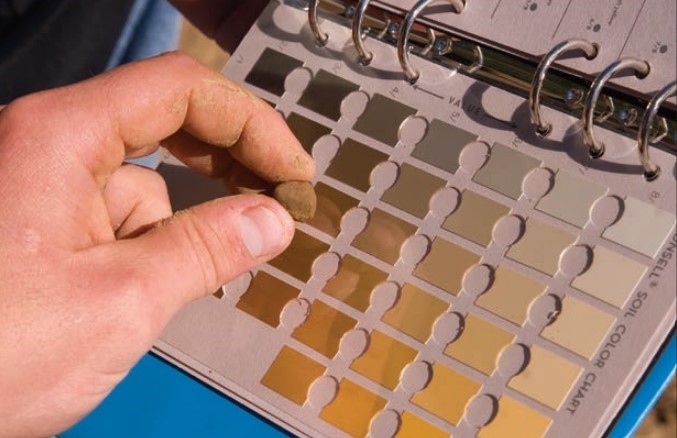
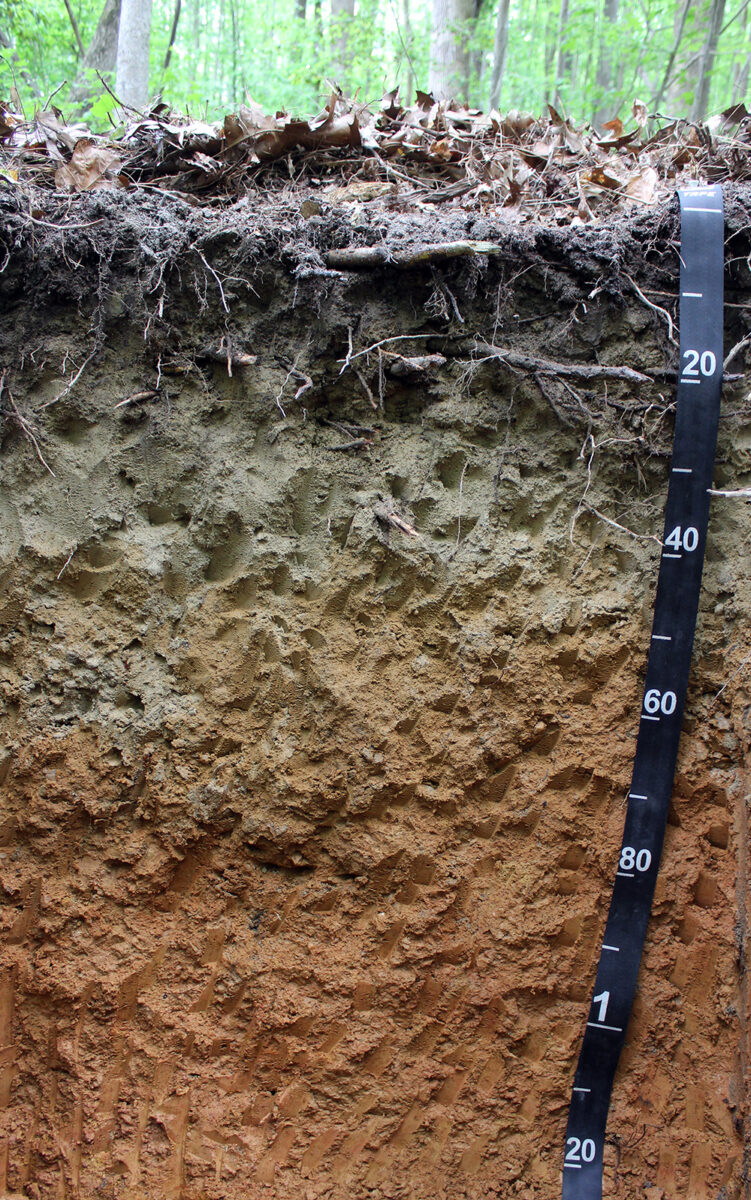
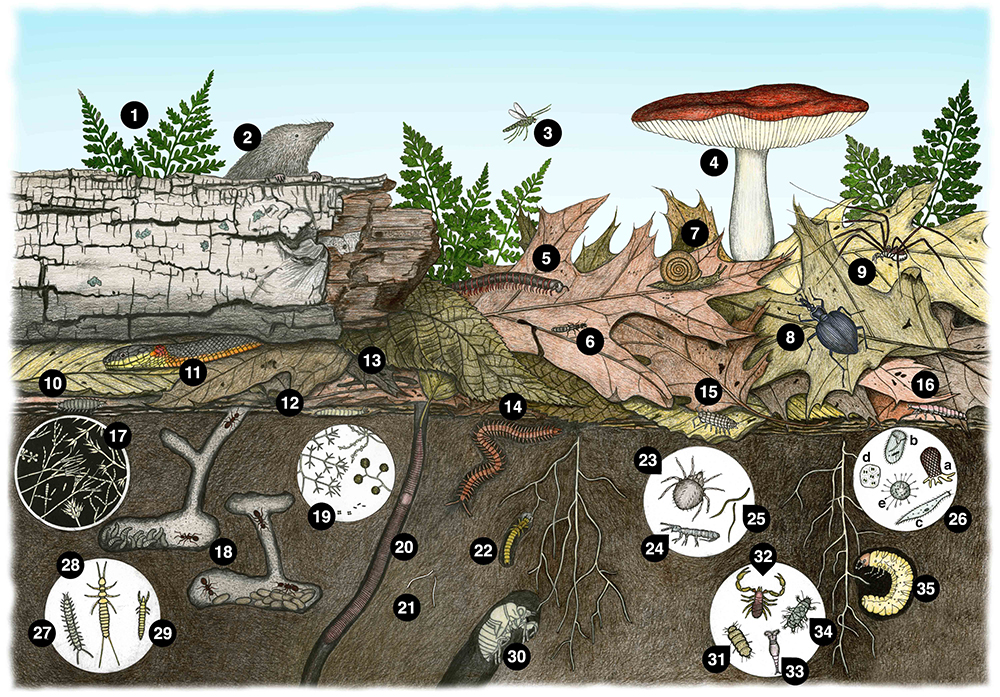
Soil is Full of Life
Beyond the nonliving mineral components of soil and the dead organic matter, soil is also composed of diverse living organisms, both fauna (animals) and flora (plants, bacteria, archaea, and fungi). These organisms are classified in multiple ways, with the simplest classifications based upon their relative size and physiology. The profiles in Chapter 6 include these groups of organisms, with brief coverage of flora and detailed focus on macrofauna and mesofauna:
- Microflora (bacteria and microscopic fungi and algae)
- Fungi (mushrooms and other macrofungi)
- Plants (mosses, lichens, liverworts, and vascular plants)
- Microfauna (protozoa)
- Mesofauna (nematodes, rotifers, tardigrades, potworms, and smaller arthropods such as springtails and mites)
- Macrofauna (larger arthropods like centipedes and beetles, isopods, mollusks, earthworms and vertebrate animals)
American Burying Beetle
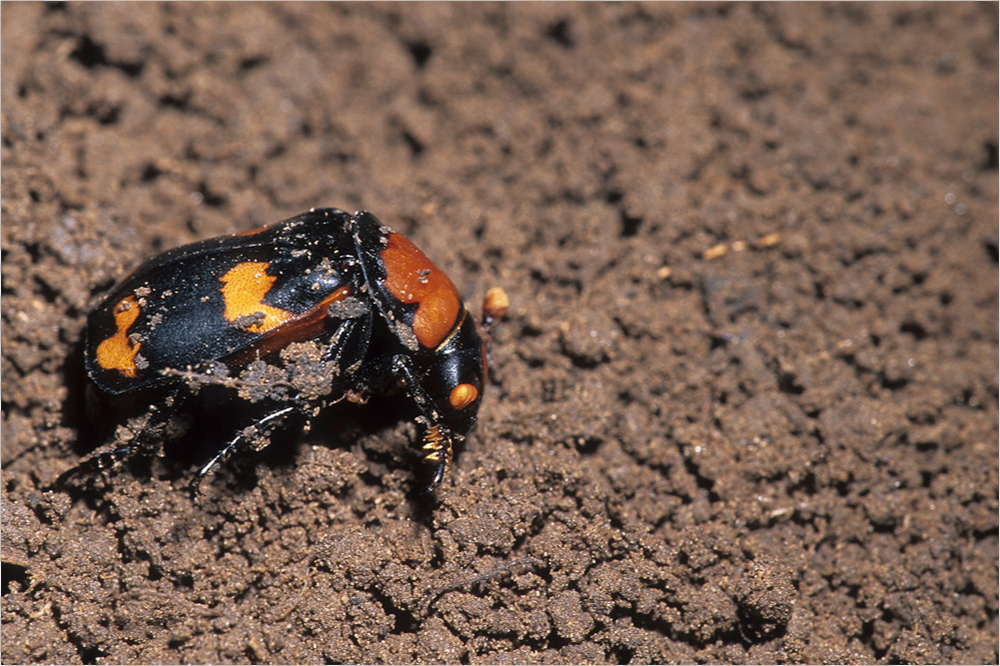
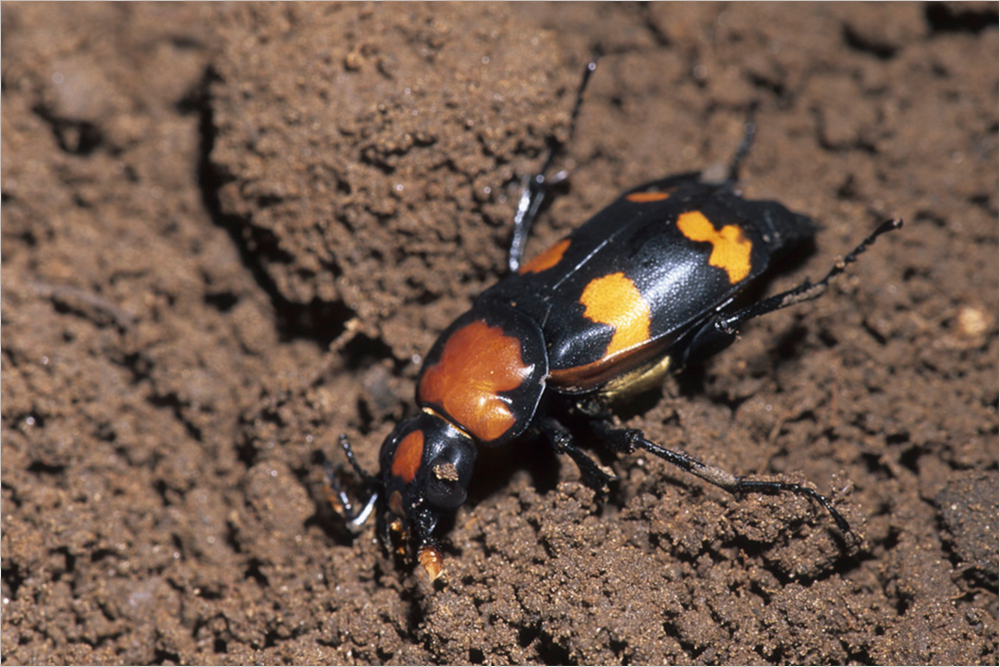
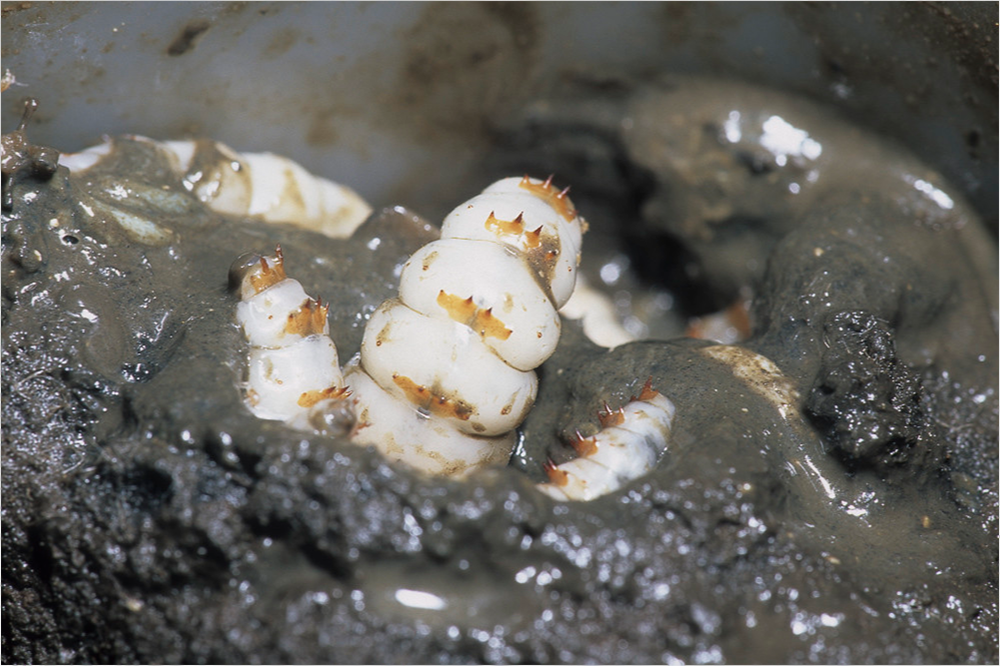
A number of beetles contribute significantly to the recycling of carrion, returning nutrients to the soil. Carrion is a small portion of detritus in most ecosystems but has an important role in nutrient cycling because it decomposes at a much faster rate than plant materials, which contain lignin, hemicellulose and cellulose—all resistant to decomposition. Carrion is also much more nutrient rich than the same amount of plant matter. Decomposing carrion creates small islands of increased soil fertility that can have long-lasting ecological effects.
Of the 31 species of burying beetles in North America, the largest is the American burying beetle (Nicrophorus americanus). At more than 1.5 in. (3.81 cm) in length, adults have shiny black bodies with bright-orange bands on their wing covers, a patch of orange between their eyes, and a dollop of orange on their antennae.
Experts at detecting the odor of death, these beetles use their antennae to help them locate small animals within an hour of death from up to two miles away (these beetles specialize in carrion the size of pigeons or small rodents such as prairie dogs or ground squirrels). A breeding pair will get to work right away to prepare the carcass.
If the ground underneath isn’t amenable (these beetles strongly prefer soils with high moisture), the beetles will move the carcass to a preferable site for burial. They will loosen the soil, plowing through it from beneath the carcass, eventually causing the carcass to settle into the ground. After covering the carcass with several inches of soil, the beetles clean off any feathers or fur and work the carcass into the shape of a ball. The female will lay eggs in a depression on top of the carcass ball. Burying beetles provide their larvae with parental care, which is uncommon in insects. The adults provide predigested carrion to the larvae on request until they have grown enough to feed themselves. American burying beetles are active at night, which prevents competition with the day-active flies that lay eggs on carcasses; the burying of the carcass also reduces competition. Thus, young burying beetles grow up in a relatively safe environment, and their work breaks down the carrion and adds nutrients to the soil. As the carrion is broken down by the beetles, nitrogen, phosphorus, potassium, sulfur, magnesium and sodium increase in soils underneath.
Once found in 35 states in the eastern and central United States, American burying beetles are now found only in undisturbed habitats (e.g., prairies, forest edges, and scrubland) in Nebraska, Rhode Island, Oklahoma, South Dakota, Kansas and Arkansas, along with populations that have been reintroduced in Ohio, Massachusetts and Missouri. The species was listed as federally endangered in 1989; in 2019, the United States Fish and Wildlife Service proposed reclassifying the beetle as threatened rather than endangered. Causes for the beetle’s decline include habitat loss and fragmentation, a reduction of the small mammals and birds on which these beetles grow, and an increase in competition with larger scavengers (e.g., crows, foxes, and raccoons) that compete for available carrion in disturbed areas. Climate change is now also a threat, since these beetles have a limited ability to tolerate warmer temperatures and changes in soil moisture.
Grouping soil organisms based upon their ecological roles is helpful for understanding their function. We do not organize animals that way in this handbook, but throughout the profiles, we describe functional groups where they apply. Here are a few examples of these ecological roles:
- Plant root symbionts (e.g., nitrogen-fixing bacteria and mycorrhizal fungi)
- Decomposers (e.g., bacteria, cellulose- and lignin-degrading fungi and earthworms)
- Elemental transformers (e.g., denitrifying bacteria)
- Soil engineers (e.g., earthworms, ants, and termites)
- Herbivores and pathogens (e.g., plant-eating nematodes, root rot fungi and root-feeding beetle larvae)
- System regulators (e.g., predatory insects, predatory nematodes, parasites and hyperparasites)
However we choose to classify them, soil dwellers represent the most species-rich community of organisms on Earth. In fact, based on some estimates, more than four million species of organisms exist in the soil (excluding plant roots), and yet we know very little about the vast majority of these life forms.
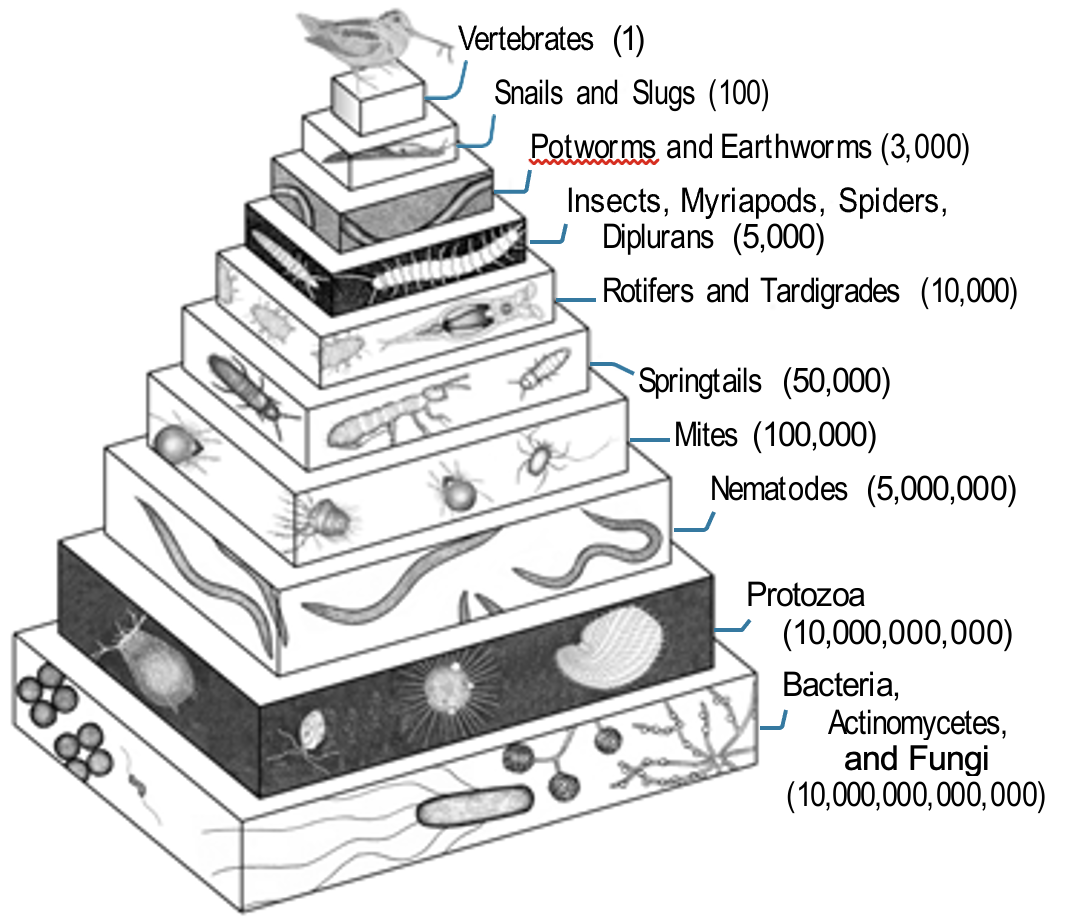
This material is based upon work that is supported by the National Institute of Food and Agriculture, U.S. Department of Agriculture through the Sustainable Agriculture Research and Education (SARE) program. Any opinions, findings, conclusions, or recommendations expressed in this publication are those of the author(s) and should not be construed to represent any official USDA or U.S. Government determination or policy.